Shaping humoral immunity to vaccines through antigen-displaying nanoparticles
March 19, 2020
Publication type
Journal Article
Summary Strategies to qualitatively and quantitatively enhance the humoral response to immunizations with protein and polysaccharide antigens are of broad interest for development of new and more effective vaccines. A strategy of increasing importance is the formulation of antigens into a particulate
format, mimicking the physical form of viruses. The potential benefits of enhanced B cell receptor engagement by nanoparticles have been long been appreciated, but recent studies are defining additional important factors governing how nanoparticle immunogens interact with the immune system in the context of lymphoid organs. This review will discuss findings about how nanoparticles enhance humoral immunity in vivo and factors governing the fate of nanoparticle immunogens in lymph nodes.
Introduction
Vaccines are a cornerstone of modern public health, and the development of vaccines against endemic pathogens has had a major impact on global mortality and morbidity from infectious diseases [1]. Many vaccines have been developed through the generation of live attenuated or chemically inactivated forms of microbes, but this approach is not always successful and in some cases (e.g. HIV) is not effective and/or safe. An alternative is to design subunit vaccines based on selected protein or polysaccharide antigens derived from the pathogen. Here the challenge becomes promoting the right type of immune response. Because nearly all available licensed vaccines are thought to protect through induction of appropriate long-lived antibody responses [2], guiding humoral immunity is a critical element in the development of subunit vaccines. Vaccine-elicited immunity is impacted by a number of factors, including the use of adjuvants, choice of immunization regimens, and structural design of the immunogen.
One particularly effective strategy for enhancing humoral responses is via formulation of antigens in a multivalent particulate structure, mimicking the structure of a virus. Nanoparticle vaccines have a number of theoretical
advantages for immune stimulation relative to soluble immunogens, including enhanced lymphatic trafficking, increased capture in lymph nodes by antigen presenting cells (APCs), and increased activation of antigen-specific B cells through receptor crosslinking [3]. Formation of particulate antigens can be achieved by fusing immunogens to a protein that undergoes self assembly with other subunits to form a nanoparticle, or
through chemical linkage to synthetic lipid vesicles, polymer particles, or other inert materials. Vaccine nanoparticles are typically designed with diameters of 100 nm or smaller, as particles in this size range exhibit effective drainage from injection sites into lymphatic vessels for enhanced trafficking to draining lymph nodes (Figure 1a) [3,4]. Particulate formulation has a demonstrated track record of efficacy in humans, as demonstrated by the licensed HPV and hepatitis B vaccines, which are based on virus-like particle immunogens.
Despite these successes, there remain many open questions about optimal design principles for nanoparticle immunogens, and how these vaccines interact with the immune system. In this review we will discuss recent advances in understanding how nanoparticle immunogens impact humoral immunity, with a particular focus on new insights into the fate of particulate antigens in vivo and how nanoparticle immunogens can be targeted to key sites for humoral immune response induction.
Factors in particulate antigen display impacting humoral immunity Display of immunogens in a dense array on the surface of a particle is often effective to increase their immunogenicity. For example, minimal peptide immunogens that elicit poor humoral responses due to rapid clearance in vivo and lack of repetitive epitopes have been demonstrated to generate
strong antigen-specific antibody responses when multimerized on a particle scaffold [5,6]. Nanoparticle display also enhances responses to T-independent immunogens, as demonstrated in the case of pneumococcal glycans; their delivery on gold nanoparticles or liposomes leads to robust
class-switched antibody responses in mice [7,8]. Nanoparticle scaffolds can also increase the stability of immunogens and maintain desired epitope conformations.
Fusion of HIV Env trimer subunits with self-assembling protein scaffolds has been successfully employed to ensure the antigen maintains the correct conformation [9,10,11,12]. Stabilizing an antigen on a particle scaffold can
also prevent off-target responses against artificial or undesirable epitopes, helping to focus the immune response on the antigenic site of interest [13]. In the case of transmembrane antigens, an engineered lipid bilayer can
be used to maintain the native conformation of an antigen that would otherwise be unstable in vivo [14,15]. Details of how the antigen is arrayed are important in shaping the humoral response to nanoparticle vaccines.
High antigen surface density appears to be important. Particles displaying antigen at a spacing allowing bivalent recognition by an individual BCR increases binding avidity (Figure1b) [10]. Using a modular multicomponent protein nanoparticle system, it was shown that antigen density correlated with neutralizing responses to an RSV immunogen in mice, with optimal particles also eliciting strong neutralizing responses in non-human primates [16].
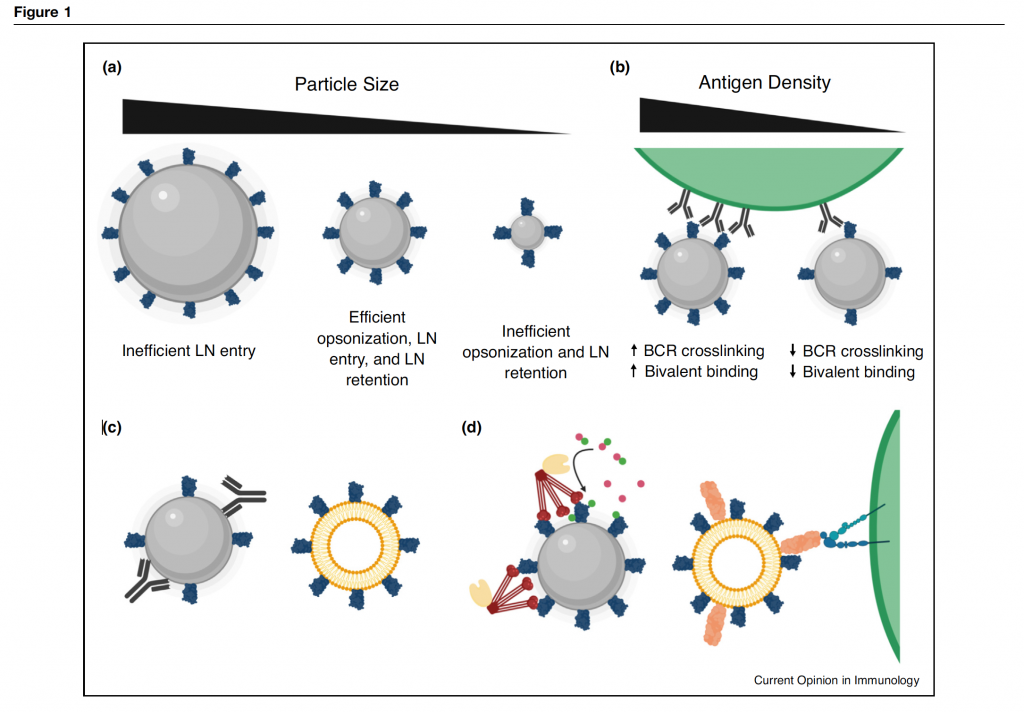
Figure 1: Physical characteristics of nanoparticles influence their interactions with the immune system. (a) Small nanoparticles (<15 nm) are poorly retained within the lymph node and are poorly opsonized, while large nanoparticles (>100 nm) are often too large to freely access the lymphatic system. (b) Increased antigen density on the surface of nanoparticles can improve both bivalent recognition by B cell receptors as well as B cell receptor crosslinking, inducing more robust B cell activation. (c) Anti-scaffold antibody responses can distract from desirable responses against the displayed antigen and can be mitigated by utilizing a poorly immunogenic particle composition. (d) Particles can engage with innate immune defense proteins to promote trafficking to FDCs. On left, mannose-binding lectin (red) can recognize surface glycans on the particle, leading to the cleavage of C3 into C3a (magenta) and C3b (green) by MBL-associated serine proteases (tan). C3b deposition on the particle then leads to directed trafficking to lymph nodes in a complement-receptor dependent manner. On right, MFG-E8 (salmon) can recognize phosphatidylserine on the surface of liposomes. The MFG-E8 can then be bound by integrins on the surface of subcapsular sinus macrophages, leading to the direct deposition of the particles on FDCs.
Similarly, dense multimerization of engineered HIV envelope trimers on liposomes has been shown to enhance triggering of antigen-specific B cells [13,17]. Nanoparticle display has also been shown to impact humoral responses in non-human primates (NHPs) [6,18]. This is particularly
highlighted by a recent NHP study of engineered HIV antigens, in which nanoparticle immunization elicited robust antibody and germinal center responses within seven days following immunization [19]. Nanoparticle carriers also enable multiple antigens to be displayed simultaneously to a single B cell. For example, presentation of multiple strains of influenza hemagglutinin in close proximity on a single nanoparticle favors the
expansion of B cells that recognize epitopes conserved between the distinct strains, promoting neutralizing anti-body breadth [20]. Sequential immunization with liposomes bearing a series of HIV Env trimers or mixtures of trimers as heterologous boosts was similarly able to elicit
antibodies exhibiting broad neutralization in rabbits [21].
A challenge particularly for protein nanoparticles is the development of strong humoral responses against the ‘core’ scaffold itself, which might immunodominate over desired epitopes on the immunogen [16,22,23]. These responses arise in part due to the use of non-self and engineered proteins to form particle cores, and incomplete particle self-assembly in some systems (Figure 1c)[12,23]. Targeting of the particle scaffold can be minimized by using particles with nonimmunogenic compositions,
such as liposomes, through engineering particle structures where the core is sterically inaccessible, or through glycan or polymer masking of the particle core. Another strategy is to design a particle composed entirely
of antigen, eliminating additional material that could
elicit an off-target response [24].
Trafficking of vaccine nanoparticles and engineering LN localization for vaccines
Effective design of nanoparticle vaccines must take into account the fate of these biomaterials in lymphoid tissues — how do they enter the lymph node parenchyma? Where do they localize and for how long? What cells and factors impact these processes? These are questions for which some answers are known and for which others remain unanswered, motivating further fundamental research to modulate immunogen trafficking for optimal vaccine-induced immunity.
Entry of particulate versus soluble antigens into lymph nodes Vaccines are typically administered parenterally. Following injection,particulate antigens with sizes greater than 5 nm are too large to enter the blood vasculature efficiently, and are instead convected into lymphatic vessels. Soluble antigens and particles less than
100 nm in diameter are efficiently trafficked through lymph to draining lymph nodes [4]. On reaching the draining node, small soluble antigens (less than 70 KDa in size) can be transported into the T cell areas or follicles ofLNs through collagen conduits [25,26], or via gaps in the floor of the subcapsular sinus [27]. Entry into the conduits is mediated by portals in lymphatic endothelial cells capped by a size filtering complex formed
by the plasmalemma vesicle-associated protein [28]. Although nanoparticles have been thought to be too large to efficiently pass the PLVP filter, recent data have shown that both vaccinia and zika virus particles can access con-
duits and penetrate deep into LNs [29].
Alternatively, particulate antigens are captured by macrophages and dendritic cells lining the subcapsular sinus (SCS) or medulla [4,30,31]. SCS macrophages express a suite of receptors enabling antigen capture, including complement receptors, Fc receptors, and CD169, and do not rapidly internalize and degrade captured particulate antigens.Particles captured by sinus macrophages are transferred over their cell surfaces or
via transcytosis to migrating follicular B cells just under the capsular floor. Dendritic cells capturing antigen in the cortical or medullary sinuses can present antigen locally or migrate into the parenchyma to initiate T and B cell priming [30,31]. I
n addition to direct lymphatic drainage, particles can also be captured in peripheral tissue by monocytes and dendritic cells, which can actively carry antigen into the LN parenchyma [4,32,33]. In addition to capture by APCs, several other mechanisms can impact antigen entry to LNs. Lymphatic endothelial cells express scavenger receptors and have been shown to capture viral particles [34]. Particulate antigens are also susceptible to rapid proteolysis in the lymph and/or SCS, and protein components of particulate antigens may rapidly reach B cells deep within follicles [34,35]. Which of the above described pathways are engaged depends on the precise composition and size of the antigen/particle and is influenced by the choice of vaccine adjuvant [36]. However, it is likely that multiple trafficking pathways occur simultaneously in parallel for any particular vaccine immunogen.
Transfer of particulate antigens to follicular dendritic cells
Once antigens have passed into the LN parenchyma, they may be trafficked to different sites with distinct implications for the ensuing immune response. For humoral immunity, localization of antigen in follicles and germinal centers (GCs), where antigen-triggered B cells undergo proliferation and somatic hypermutation, is critical. Follicular dendritic cells (FDCs) play an important role in GCs, by presenting immobilized antigens to B cells for extended periods [37]. FDCs are primarily thought to
present antigen on their dendritic surfaces via Fc and complement receptors (CR1 and CR2), and mice deficient in CR1/2 exhibit substantially impaired antibody responses to vaccination [38,39]. Thus, delivery of vaccine immunogens to FDCs is an important step for effective humoral immunity.
Nanoparticles and immune complexes can be transportedto FDCs in a complement-dependent manner. Complement (C) opsonization occurs via one of 3 pathways: the classical pathway involving IgM or IgG bound to the
particle, the lectin pathway involving mannose binding lectin (MBL) recognizing sugars on the particle surface, or via spontaneous deposition of complement on the particle surface through the alternative pathway [40]. SCS macrophages capturing C-opsonized particles transfer the antigen to non-antigen-specific B cells migrating near the lymphatic sinuses, which subsequently deliver these antigens to FDCs in a CR1/2-dependent manner [4]. In addition to complement-mediated trafficking, other pathways for transport of particulate antigens to FDCs have been described. Medullary DCs have been shown to capture influenza viral particles via the lectin receptor SIGN-R1, and carry them toward FDCs [30], although it remains unclear whether these DCs transport antigen all the way to FDCs or transfer to B cells occurs at some intermediate point. Recently, a pathway for direct transfer of enveloped viral particles to FDCs was identified, whereby the phosphatidyl serine-binding protein MFGE8 acts as an adaptor binding viral membranes to integrins on SCS macrophages, which can then directly transfer viral particles to abutting FDCs [41]. Hence, a number of overlapping mechanisms exist to efficiently transport particulate antigens to the FDC network.
Engineering particulate immunogens to alter in vivo trafficking
As noted in the discussion above, administration of immunogens in a nanoparticle as opposed to soluble/monomeric format immediately alters LN trafficking and localization. This is clearly illustrated in studies of the LN
localization of soluble versus particulate HIV Env immunogens. Soluble HIV gp120 or gp140 trimer immunogens localize to interfollicular regions, concentrated on SIGN-R1-expressing macrophages that recognize sugars on
these heavily glycosylated immunogens [12,42]. By contrast, nanoparticle forms of gp120 or Env trimers were recently shown to concentrate on FDCs within a few days of immunization, and colocalize with nascent GCs [12]. This FDC localization was mediated by MBL-mediated recognition of the dense glycan coat of these NP immunogens, triggering complement deposition and subsequent CR1/2-dependent trafficking into follicles (Figure 1d). MBL recognition of glycosylated nanoparticles increased serum antibody, GC B cell, and plasma cell responses to vaccination. A similar pattern of localization within follicles could be engineered for inert polymer nanoparticles by chemically conjugating a threshold density of minimal trimannose glycans onto particle surfaces, suggesting that nanoparticles can be engineered to improve antigen delivery to FDCs [12].
In a similar manner, the recently described MFG-E8-mediated transfer of viral particles to FDCs might be engineered into liposomal vaccines [41].
Particle size is a second key parameter (Figure 1a). Recent systematic studies using gold nanoparticles surface-conjugated with the model antigens showed that access to follicles is strongly impacted by particle size.
Nanoparticles <15 nm in diam. rapidly entered LN inter-follicular regions, the SCS, and follicles following immunization, but cleared from follicles by 48 hours [43]. By contrast, larger 50 or 100 nm diam. antigen-conjugated
particles were largely confined to the SCS at 2 hours post immunization, but were trafficked into follicles by 12 hours and persisted there for several weeks in a C3 and complement receptor-dependent manner. Histological
staining confirmed that these follicle-localized particles colocalized with FDCs and GCs at later times. TEM imaging revealed that the small nanoparticles were predominantly endocytosed by FDCs, while larger particles were immobilized on the extracellular surface of FDCs,
suggesting effective localization for antigen acquisition
by cognate B cells [43].
The inclusion of an adjuvant, either as a component of the nanoparticle or as an additional surface functionalization, is a third approach to humoral immunity [44–47], and adjuvant-driven inflammation alters the lymph node in diverse ways that can impact antigen trafficking. For example, it was recently shown that UV-inactivated influenza nanoparticle vaccines internalized by lymph node macrophages trigger an inflammasome-mediated, necrotic-like cell death, promoting a pro-inflammatory cytokine and chemokine cascade in responding lymph nodes while simultaneously removing these macrophagesas potential antigen transfer agents [48]. This mechanism could potentially be active in many nanoparticle vaccines as inflammasomes are activated in response to a wide variety of nanoparticle compositions.
Conclusions
Recent studies in both small and large animal models are providing new insights into the impact of particulate formulations of vaccine antigens on humoral immunity. While much effort has focused on engineering antigen
display to optimally present neutralizing epitopes, an equally important component will be consideration of engineering nanoparticle designs to exploit natural pathways for optimal entry and localization within lymph
nodes. Studies to further our understanding of how the immune system processes particulate antigens should help define improved design strategies for future vaccines.
Conflict of interest statement
Nothing declared.